1. 引言
自然界的生物普遍进行着呼吸运动,作为最高级动物的人,更是以呼吸为基础进行着维持生命的新陈代谢活动。呼吸运动是一种节律性的运动,其产生部位和产生机理一直是神经科学研究领域的重要课题。1991年的一项呼吸系统生物实验发现,位于哺乳动物脑干中的一个特殊区域——前包钦格复合体(pre-Bötzinger complex)可能是呼吸节律起源的关键部位 [1] ,而且前包钦格复合体中神经元一些特殊的放电行为是产生呼吸节律变化的重要因素。为了探究呼吸产生的机制,人们对这种放电行为进行大量动力学研究。
1991年,Butera等人为pre-BötC中的单个和耦合神经元细胞分别建立了基于持续钠电流(
)的数学模型 [2] [3] 。Toporikova和Butera提出了一个pre-Bötzinger神经元中的双腔室电导模型(TB模型),结果表明此模型可以产生依赖于持续钠电流(
)和钙激活非特异性阳离子电流(
)的神经元放电 [4] [5] [6] 。随后,Park和Rubin对TB模型进行了简化处理,提出了一个pre-BötC的单腔室电导模型,此模型可以和双室模型产生同样的放电类型 [7] 。Rinzel率先使用快慢变量分离和分岔分析的动力学分析方法,为人们后续理解神经元放电活动的产生机理建立了科学依据 [8] [9] [10] [11] 。Dunmyre等人也提出了混合放电的产生机制的新解释方法 [12] 。此外,Wang和Rubin等人还观察到了混合放电(MB),并且对胞体–树突簇放电(S-D Bursting)的参数区域做出了分类 [13] 。Duan等人研究了耦合神经元的同相簇放电和反向簇放电的放电模式,并对其进行了分类 [14] [15] 。Wang等人研究了耦合pre-BötC模型中混合放电的动力学机制 [16] 。随着研究的深入,Lü和Chen等人在单个pre-Bötzinger神经元中发现了大量混合放电模式,对其产生进行动力学分析并将其分类 [17] [18] 。这些研究成果为我们研究呼吸系统神经元混合簇放电提供了重要帮助,但影响胞体–树突簇放电的因素还有很多,需要我们逐步完善。
本文主要研究了在持续钠电流和钙激活非特异性阳离子电流影响下,pre-Bötzinger复合体神经元中胞体–树突簇放电的转迁机制。第一部分主要介绍pre-Bötzinger复合体的单个神经元的动力学模型;第二部分主要讨论钙系统中的重要参数
和钙激活非特异性阳离子电导(
)对胞体–树突混合簇放电的影响并解释其产生机制;第三部分主要讨论持续钠离子电导对胞体–树突混合簇放电的影响;在文章的最后给出结论。
2. 模型介绍
这里我们研究的模型为pre-Bötzinger复合体的单个神经元模型 [7] ,探究该模型产生的胞体–树突簇放电(S-D Bursting)以及其蕴含的动力学机制。该模型的具体描述如下:
(3-1)
(3-2)
(3-3)
在上式中,C是膜电容,V是膜电位,n和h分别为钾通道和钠通道的门控变量。
、
、
、
、
分别表示钠离子电流、持续钠离子电流、泄漏电流、钾离子电流和钙激活非特异性阳离子电流。上述各离子电流的模型和电压门控函数分别为:
钙离子相关函数表达式为:
(3-4)
(3-5)
其中,
是指细胞内Ca2+浓度,
是从内质网(ER)流入细胞质的通量,
是从细胞质流出到内质网的通量,
是胞浆中游离的Ca2+浓度的比例常数。
由
和
共同控制,且通过
来调节。l是内质网膜上未被激活的
通道所占比例,由
决定。其中,
和
的大小和细胞内
浓度以及控制
通道的门控变量l有着不小的联系,具体表达式如下:
(3-6)
其中,
是三磷酸肌醇,
和
是内质网泄漏渗透性和最大渗透性,
是
所需的
受体激活的解离常数,
是所需的
受体激活解离常数,
是细胞溶质和内质网体积之比。
系统(3-1)~(3-5)为全系统,其中系统(3-1)~(3-3)为胞体子系统,系统(3-4)~(3-5)为树突子系统。在全系统中,树突子系统独立于胞体子系统,但是胞体子系统受树突子系统影响。系统中的其他参数值在附录中显示。
3. 钙激活非特异性阳离子电流对胞体–树突混合簇放电的影响
去甲肾上腺素(NE)是调节pre-Bötzinger复合体中神经元呼吸节律的重要因素 [19] [20] 。去甲肾上腺素通过控制钙激活非特异性阳离子电流(
)来影响胞体–树突簇放电的放电模式 [19] [20] ,而
受
和
的共同影响,所以我们变化
和
来研究胞体–树突混合簇放电的变化规律。
3.1.
变化对胞体–树突混合簇放电的影响
在这节中我们固定
和
。当
时,全系统出现了如图1所示的胞体–树突混合簇放电。图中黑色实线表示膜电位的时间序列,红色划线表示细胞内钙离子浓度
的时间序列。从图中可以看出,
有两个状态,一个是缓慢增加的状态,产生的簇放电为胞体簇放电;而另一个跳跃的状态,产生的簇放电为树突簇放电。树突放电是受胞体放电和
振荡跳跃共同影响,所以我们重点研究胞体部分来考虑
对胞体–树突簇放电的影响。
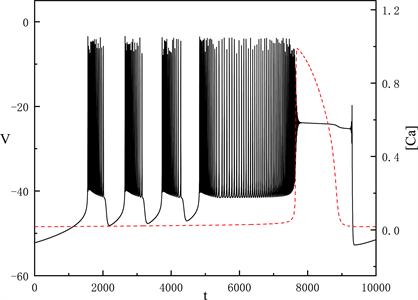
Figure 1. Time series of the membrane potential V with
图1.
时,膜电位V的时间序列
图1中显示的胞体放电分为两种类型,我们将利用快慢变量分离和分岔分析的方法,对这两种放电类型进行分析。我们将h当作慢变量,固定每段胞体放电中
的平均值,得到胞体中每段放电模式对应的快子系统关于慢变量h的分岔图,如图2和图3所示,两段胞体放电分别为“fold/homoclinic”型簇放电和峰放电。
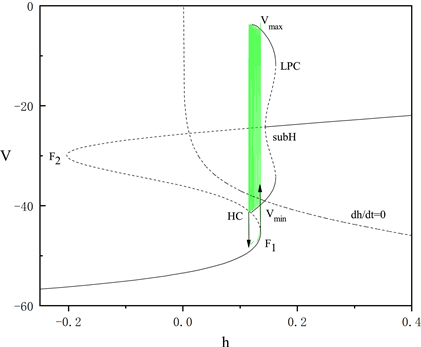
Figure 2. The fast-slow decomposition and bifurcation analysis of the first bursting in the whole system with
and
fixed
图2.
时,固定
,全系统中第一段簇放电的快慢分解和分岔分析
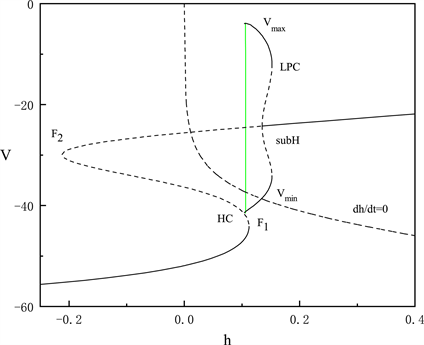
Figure 3. The fast-slow decomposition and bifurcation analysis of the last spiking in the whole system with
and
fixed
图3.
时,固定
,全系统中后一段峰放电的快慢分解和分岔分析
图2和图3为分岔图在
平面上的投影,其中绿色曲线表示全的轨迹。由于胞体前部的三段短簇类型一致,我们只讨论第一段短簇的类型,将
固定为0.019。图2中平衡点组成了一条S形曲线,鞍结分岔点
和
将该曲线分为上中下三支。曲线的上支由稳定和不稳定的焦点组成,中间分支为鞍点,下分支为稳定的结点。上支中稳定和不稳定的焦点由亚临界霍普夫分岔点(subH)分隔,并从该点分岔出一个不稳定的极限环。该极限环的最大振幅值为
,最小振幅值为
,在同宿轨分岔点(HC)处消失。极限环上的鞍结分岔(LPC)使极限环上的不稳定点转化为稳定点。簇放电的方向由h零等值线(
)决定。系统轨线通过鞍结分岔点(
)由静息态转变为放电态,又通过同宿轨分岔点(HC)转变为静息态的簇放电的类型被称为“fold/homoclinic”型簇放电。
当
固定为0.028时,对胞体后段的放电模式进行了快慢变量分离和分岔分析。图3中显示胞体后段的放电类型为峰放电。
随着
不断增加,当
时,胞体部分的放电类型产生了很大变化,如图4所示。
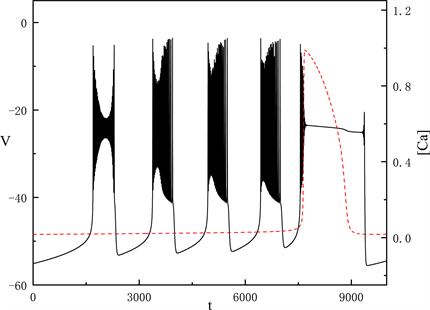
Figure 4. Time series of the membrane potential V with
图4.
时,膜电位V的时间序列
我们讨论胞体部分的第一段簇放电,固定
,图5中快子系统在亚临界霍普夫分岔点(subH)处产生了极限环上的鞍结分岔(LPC)。系统轨线经由鞍结分岔点(
)跃迁到曲线上支,经亚临界霍普夫分岔点(subH)由静息态转变为放电态,又经亚临界霍普夫分岔点(subH)转变为静息态。这种簇放电模式被称为经由“fold/homoclinic”滞后环的“subHopf/subHopf”型簇放电。
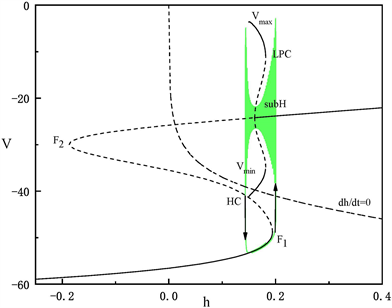
Figure 5. The fast-slow decomposition and bifurcation analysis of the first bursting in the whole system with
and
fixed
图5.
时,固定
,全系统中第一段簇放电的快慢分解和分岔分析
由于胞体部分的后三段簇放电类型相同,在这里我们只讨论第二段簇放电的类型。固定
,如图6所示。系统轨线的静息态经由鞍结分岔点(
)跃迁到曲线上支,经由亚临界霍普夫分岔点(subH)转变为放电态,又经同宿轨分岔(HC)转变为静息态。我们将这种簇放电模式称为经由“fold/homoclinic”滞后环的“subHopf/homoclinic”型簇放电。
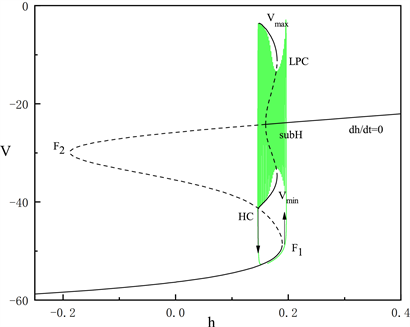
Figure 6. The fast-slow decomposition and bifurcation analysis of the second bursting in the whole system with
and
fixed
图6.
时,固定
,全系统中第二段簇放电的快慢分解和分岔分析
上面我们分析了两种由
变化产生的胞体–树突混合簇放电类型。图7中显示了
的变化对胞体–树突混合簇放电的影响。首先,当
时,胞体部分放电类型为峰放电。当
逐渐增加到0.096 μM时,胞体的前部产生了一个“fold/homoclinic”型簇放电,后部仍然为峰放电。随着
的增加,胞体前部的短簇逐渐增多,当
时,胞体中的簇放电的类型变多。胞体前段的第一个簇放电为“subHopf/subHopf”型簇放电(经由“fold/homoclinic”滞后环),胞体中其他的簇放电为“subHopf/homoclinic”型簇放电(经由“fold/homoclinic”滞后环)。随着
的增加,胞体中的第二个簇放电由“subHopf/homoclinic”型簇放电(经由“fold/homoclinic”滞后环)变为“subHopf/subHopf”型簇放电(经由“fold/homoclinic”滞后环)。
的增加不改变全系统簇放电的周期,但减少了全系统放电的持续时间。
3.2.
变化对胞体–树突混合簇放电的影响
我们固定
,
,将
从0.6 nS逐渐增加到1.2 nS,pre-Bötzinger复合体中神经元产生的胞体–树突簇放电(S-D Bursting)模式的变化如图8所示。图中左侧纵轴表示膜电位V,右侧纵轴则表示钙离子浓度
。图中红色划线为
的时间序列图。全系统簇放电的胞体部分对应于钙离子浓度
变化缓慢的部分,树突部分对应于
变化迅速的部分。
全系统中出现了胞体–树突混合簇放电(如图8)。胞体的簇放电类型为“subHopf/subHopf”型簇放电(经由“fold/homoclinic”滞后环)。随着
的增加,胞体前部的短簇数量减少,直到
时,胞体–树突混合簇放电前部中的所有放电模式均变为峰放电。
的增加导致胞体混合簇放电的持续时间增加,但不改变胞体部分和树突部分的周期。
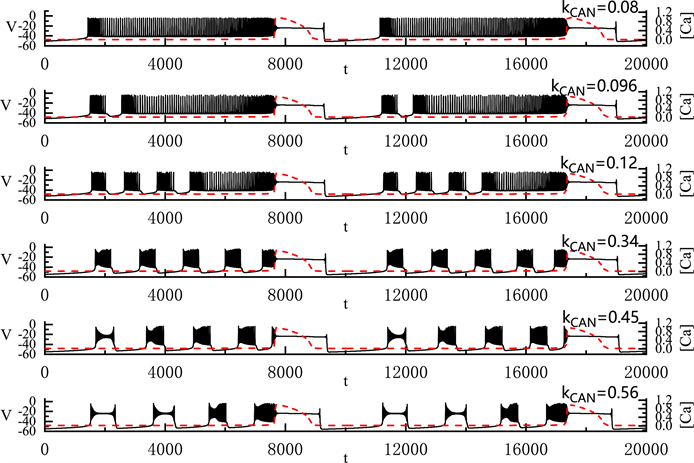
Figure 7. Variation of the somatic-dendritic mixed bursting with
and 0.56 μM
图7. 胞体–树突混合簇放电的类型随
的变化情况。
取值从上到下依次为0.08, 0.096, 0.12, 0.34, 0.45和0.56 μM
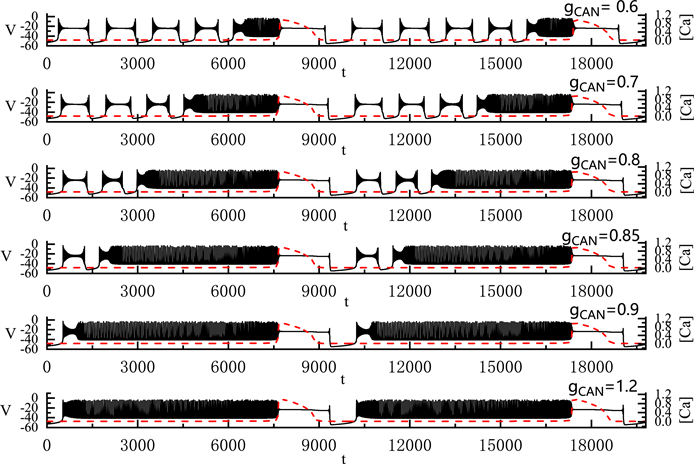
Figure 8. Variation of the somatic-dendritic mixed bursting with
and 1.2 nS
图8. 胞体–树突混合簇放电随
的变化情况。
取值从上到下依次为0.6, 0.7, 0.8, 0.85, 0.9和1.2 nS
4. 持续钠离子电流对胞体–树突混合簇放电的影响
研究发现,持续钠电流的变化会导致细胞缺血缺氧 [21] ,进而影响神经元的呼吸节律。实验发现增大持续的钠电流,细胞的动作电位幅度和频率也会增加,同时持续钠电流的小幅度增大可以引起细胞动作电位的时程明显增加 [22] 。持续钠电流的幅度增大导致细胞内Na+浓度增高,进而引起细胞内Ca2+浓度增高以及细胞兴奋性改变 [21] [23] 。因此我们变化持续钠离子电导(
)来研究pre-Bötzinger复合体中胞体–树突混合簇放电的变化规律。
我们固定
,
,将
从4.5 nS逐渐增加到12.8 nS,pre-Bötzinger复合体中神经元产生的胞体–树突混合簇放电模式如图9所示。
全系统中出现了胞体–树突混合簇放电(如图9)。当我们将
取值4.5 nS时,胞体前部的簇放电类型为经由“fold/homoclinic”滞后环的“subHopf/subHopf”型簇放电,胞体中其他簇放电为经由“fold/homoclinic”滞后环的“subHopf/homoclinic”型簇放电。随着
的增加,胞体中经由“fold/homoclinic”滞后环的“subHopf/homoclinic”型簇放电逐个变为经由“fold/homoclinic”滞后环的“subHopf/subHopf”型簇放电。直到
时,胞体部分的所有簇放电均变为经由“fold/homoclinic”滞后环的“subHopf/subHopf”型簇放电。与之前的结论一致,
的增加使胞体混合簇放电的持续时间增加,但不改变全系统的周期。
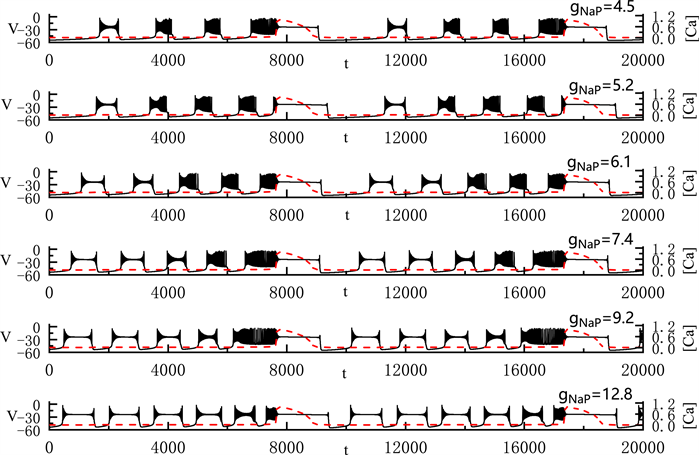
Figure 9. Variation of the somatic-dendritic mixed bursting with
and 12.8 nS
图9. 胞体–树突混合簇放电随
的变化情况。
取值分别为4.5, 5.2, 6.1, 7.4, 9.2和12.8 nS
5. 结语
在pre-Bötzinger复合体模型中,我们分析其中几种重要的影响因素——钙激活非特异性阳离子电导
和
以及持续钠离子电导
,观察他们对胞体–树突簇放电(S-D Bursting)模式的影响。从结果来看,系统中产生了不同类型的胞体–树突混合簇放电,且这些参数都对各种混合簇放电产生了各自不同的影响。
的增加导致胞体–树突混合簇放电的类型增加,由峰放电变为“fold/homoclinic”型簇放电,再变为“subHopf/subHopf”型簇放电(经由“fold/homoclinic”滞后环)。
的增加导致系统胞体前部的经由“fold/homoclinic”滞后环的“subHopf/subHopf”型簇放电的数量减少直至变为峰放电。
的增加导致系统胞体部分的短簇类型从经由“fold/homoclinic”滞后环的“subHopf/homoclinic”型簇放电逐个变为经由“fold/homoclinic”滞后环的“subHopf/subHopf”型簇放电且数量增多。这几个参数的增加都改变了簇放电的持续时间,但不改变混合簇放电的周期。胞体–树突混合簇放电的种类比较丰富,可以显示出更多的神经信息。我们对于这类混合簇的研究逐渐深入,有助于揭示呼吸运动节律的起源和产生机制,为我们进一步探究呼吸运动的其他方向打下坚实基础,势必将极大地提高人类的健康水平,对生命事业的进步产生一定的推动作用。
附录